Maximizing
Signal Strength for OFDM Inside Buildings
|
Electrical and Computer Engineering
James Cook University, Douglas Campus, Townsville, Queensland,
4814, Australia
Email: ofdm@skydsp.com,
Keith.Kikkert@jcu.edu.au |
Published: E.
Lawrey, C. J. Kikkert, "Maximising Signal Strength
of OFDM inside buildings", IEEE Transactions on
Microwave Theory and Techniques, Nov 2001, pp. 2131-2136
|
IEEE retains the copyright to this paper, which can be found
at www.ieee.org/copyright/
Abstract
Propagation inside buildings suffer from large shadowing
and high multipath effects. This is a serious problem for
Wireless Local Area Network (WLAN) systems. This paper shows
that shadowing and path loss can be minimized by exploiting
the multipath tolerance of Orthogonal Frequency Division Multiplexing
(OFDM). This can be achieved by using multiple transmission
antennas spread over the area of a WLAN cell. These antennas
act as repeaters, transmitting and receiving the same signal
at the same time. This decreases the average path loss, but
increases the multipath delay spread. Using OFDM allows the
advantage of reduced path loss to be utilized without detrimental
effects of Inter-Symbol Interference (ISI) caused by the increased
delay spread. The reduced path loss allows an increased system
capacity, quality of service or a decrease in intercellular
interference in a cellular WLAN.
Index terms-- Access point repeater, broad-band communications,
indoor radio communication, multipath channels, orthogonal
frequency division multiplexing, wireless LAN.
I. Introduction
Wireless networking is an emerging technology allowing users
the freedom of movement. The aim of wireless local area network
(WLAN) systems is to provide users with a data rate comparable
with wired networks within a limited geographic area. Currently
most WLAN products use direct-sequence spread-spectrum (DSSS)
techniques, based on the IEEE 802.11b Standard, providing
a data rate of 11 Mb/s in the 2.4- GHz industrial-scientific-medical
(ISM) band [1]. The next generation of WLAN systems will be
based on two similar WLAN standards known as: Hiperlan/2 (Europe),
and IEEE802.11a (U.S.) [2]. These support a physical layer
transmission rate of up to 54 Mb/s and use orthogonal frequency-division
multiplexing (OFDM) for the physical layer implementation.
OFDM is a multi-carrier modulation scheme, which has a high
immunity to multipath effects and allows a wide range of carrier
modulation schemes to be used. Hiperlan/2 adaptively changes
the forward error correcting coding rate and the carrier modulation
scheme (BPSK, QPSK, 16 quadrature amplitude modulation (QAM),
64 QAM) allowing the data rate to be maximized based on the
current radio channel characteristics. Using a higher spectral
efficiency modulation scheme, such as 64 QAM, allows the data
rate to be increased, but requires a higher SNR for a fixed
error rate. Thus, minimizing the path loss, allows the SNR
and corresponding data rate to be maximized.
Shadowing can result in numerous regions of the building
having an inadequate coverage, resulting in a poor quality
of service (QOS). This is a significant problem as the QOS
of WLAN systems is very important if they are to replace wired
networking. Shadowing also increases the average path loss
compared with free space, requiring more transmission power,
in order to maintain communications.
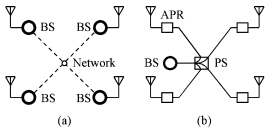
Fig. 1, Methods for obtaining spatial diversity.
Access point repeater (APR). Base station (BS). Power splitter/combiner
(PS). (a) Cellular system with four cells controlled by four
BSs, connected with a wired network for hand off between cells.
(b) Single multiple transmission cell using APRs.
High-quality coverage of a building can be obtained by using
a cellular system as shown in Fig. 1(a). Each base station
(BS) forms a cell covering a small area of the building. These
cells operate at different frequencies to prevent interference
between them. The path loss is minimized, as the distance
from the mobile station to the closest BS is minimized. Additionally
if the transmission path to the closest BS is blocked, the
mobile can connect to another cell. However, this type of
implementation can be expensive, due to the requirement of
a number of BSs. Additionally, it requires multiple frequency
bands for cellular implementation and hand offs between cells,
increasing the system complexity. This paper presents a simpler
and cheaper method for obtaining reliable coverage, in an
environment that suffers from shadowing.
II. Spatial Diversity by Multiple Repeat Transmissions
A form of spatial diversity can also be achieved by splitting
the signal from a single central BS and transmitting the same
signal from multiple locations around the building to be covered.
This is shown in Fig. 1(b). Shadowing is reduced because there
are multiple opportunities to receive the signal. Even if
several paths are blocked by walls or buildings, others may
have a reasonable transmission path. Using multiple transmitters
effectively reduces the transmission distance, as the closest
transmitter dominates the signal power.
An example of how spatial diversity maximizes power efficiency
is lighting of a building. If you were trying to illuminate
a typical large building with a single light bulb, the high
opacity of the walls would result in many deep shadowed regions.
The amount of power that would be required, for all parts
of the building to be reasonably bright, would be extremely
high, as shadowed regions would be lit solely from reflections
and gaps in obstructing walls, such as doorways. However,
by using one light per room, the illumination will be much
more even, with minimal shadowing and a reduced total lighting
power. The same is also true for radio signals; thus by using
multiple transmitters spread over the area of a building,
we can reduce the average path loss.
Most communication systems need two-way communications using
a forward and reverse link. Due to the reciprocal nature of
radio propagation, the properties of the reverse link will
be the same as the forward link. Thus, the path loss for the
reverse link can also be minimized by using multiple receive
antennas connected to a single central BS. The receive and
transmit antennas can be combined into one unit, referred
to here as an access point repeater (APR). The use of APRs
can provide a significant improvement with minimal expense.
The problem with using APRs is that the mobile receiver will
see signals from all APR transmitters. The propagation delay
from each transmitter will be different and, thus, will arrive
at the receiver with a different delay. This is equivalent
to receiving the signal distorted by strong multipath. This
causes frequency-selective fading, and an increase in the
delay spread of the transmission, which can result in inter-symbol
interference (ISI). This is particularly a problem for modulation
schemes such as frequency shift keying (FSK), as the maximum
symbol rate is limited by ISI caused by delay spread.
DSSS systems have a high multipath tolerance due to use of
a RAKE receiver [3]. A RAKE receiver uses correlation to resolve
delayed copies of the signal caused by multipath propagation.
These are then aligned in time and combined. This allows the
signals from each of the transmitters to be resolved and combined.
The maximum delay spread that can be tolerated by an IEEE802.11b
DSSS WLAN system, using a 16-tap RAKE receiver, is 125 ns
at 11 Mb/s and 250 ns at 5.5 Mb/s [4]. This level of multipath
tolerance should allow APRs to be spaced out with a maximum
diameter of about 20-35 m, although problems might arise due
to the increased delay spread. This small antenna spacing
means that there would be little advantage in using multiple
APR with an IEEE802.11b system as sufficient coverage can
easily be obtained over 20-35 m with a single transmitter.
OFDM has a higher multipath tolerance than DSSS, and can
transmit using a higher spectral efficiency. Minimizing the
path loss allows the SNR to be increased, provided inter-cellular
interference is low. This improved SNR can be utilized by
adaptively setting the modulation scheme based on the SNR,
allowing the throughput of the system to be increased.
The high multipath tolerance of OFDM is due the low symbol
rate used, and the use of a guard period between symbols.
The guard period is a cyclic extension of each OFDM symbol,
giving protection against ISI, provided it is longer than
the delay spread of the radio channel. Typically, the guard
period is made to be a small fraction of the OFDM symbol time
and, thus, by using a large number of narrow bandwidth carriers,
the delay spread tolerance can be varied to suit the radio
conditions.
Multipath causes frequency-selective fading, which can result
in carriers of the OFDM signal being lost in nulls in the
spectrum. These nulls are typically handled by including forward
error correction to compensate for the lost data. Adding multiple
transmitters will result in an increased delay spread, resulting
in a decrease in the correlation bandwidth of the channel.
This will not cause any detrimental effects on the OFDM transmission,
provided that enough carriers are used.
Indoor propagation normally suffers from a combination of
Rician and Rayleigh fading [5]. Using multiple APRs will increase
the multipath energy, resulting in more areas in the building
suffering from Rayleigh fading. However, this increase in
fading should be more than compensated for by the decreased
path loss due to the multiple APRs. More research is needed
to establish the extent of the additional multipath when using
APRs.
The use of multiple transmitters to obtain improved coverage
was first introduced in the digital audio broadcasting (DAB)
system [6]. DAB uses OFDM with a low symbol rate and a long
guard period. With DAB, it is possible for all transmitters
to use the same frequency and transmit copies of the same
signal, referred to as a single-frequency network (SFN). For
VHF band transmissions, DAB uses 1536 carriers with a the
symbol time of 1 ms, and a guard period of 246 ms, allowing
transmitters to be up 96 km apart in a SFN, before the delay
spread is too large.
Hiperlan/2 has a guard period of 800 ns, which would provide
an effective delay spread protection of up to 250 ns. This
would allow APRs within each cell, to be placed with a maximum
diameter of 40-60 m, which is sufficient for coverage of most
buildings. Additional research is needed to verify this.
III. Implementation of an APR
All APRs within the same cell transmit and receive the same
signal, thus only one BS is needed per cell. For transmission,
the signal generated by a BS is split N ways, where N is the
number of APRs. Coaxial cable or some other medium, such as
optic fibre, is used to deliver the signal to each APR. The
signal then is amplified at the APR to compensate for any
losses in the coax transmission. For reception, the reverse
process is used. Each APR has a low noise amplifier (LNA)
to compensate for losses in the coax, in order to maintain
a low noise figure. The received signals from all APRs are
combined, then demodulated at the BS. Phase differences between
the APRs have little or no effect as they corrected for, in
the OFDM demodulation process.
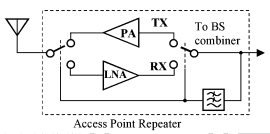
Fig. 2. Possible implementation of an APR.
Fig 2. shows a simple implementation of an APR, which can
be used when the loss in the coaxial cable is low (< 20 dB).
The master head amplifier operates at the RF frequency and,
thus, no frequency translation is required. A low frequency
control signal can be used to select whether to transmit or
receive. For a full duplex system, the RF switches can be
replaced with diplexers.
In larger systems where the cable losses are too large, the
signal can be transmitted on the cable at an IF frequency.
A common clock reference must be used by all APRs to ensure
frequency synchronization, otherwise frequency errors will
reduce the orthogonality of the OFDM, causing inter-carrier
interference. A suitable out-of-band clock reference must
be transmitted from the base station. This common clock can
then be frequency multiplied using a phase-locked-loop (PLL)
to generate the local oscillator (LO) for the IF-RF mixing.
For such a design, care must be taken to ensure the phase
noise of the clock reference is low and that the clock signal
does not interfere with the received signal.
Another method for distributing high frequency RF signals
is to use optic fiber. The RF signals can be converted to
optical signals and transmitted over long distances with optic
fiber. This type of technology is currently being developed
for use in mobile phone applications as a method for producing
pico-sized cells [7].
IV. Experimental Setup
An experiment was set up to test the effectiveness of using
multiple transmitters (i.e., APR) to minimize shadowing in
an indoor environment. The forward link from the BS to the
mobile stations was tested, by measuring the effective path
loss from fixed transmitters to a mobile receiver. The path
loss from a single transmitter (simulating a single access
point) was compared with the path loss when using two transmitters
(simulating two APRs).
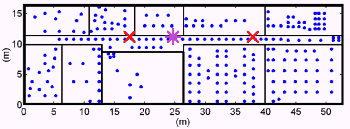
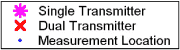
Fig. 3, Path-loss measurement locations in
the ECE building. Lines represent internal walls. The thin
lines are plaster board walls, and the thick ones are concrete
walls.
The path loss was measured at 235 locations on the second
floor of the Electrical and Computer Engineering (ECE) building
at James Cook University, Townsville, Qld., Australia. The
measurement locations and the building layout are shown in
Fig. 3. The internal walls are shown as dark lines. The selection
of the measurement locations, the position of the transmitters,
and the construction of the building can easily influence
the overall path-loss probability distribution. However, the
results presented will be representative of the improvement
that could be expected in a typical building of similar construction
to the one the measurements were taken in.
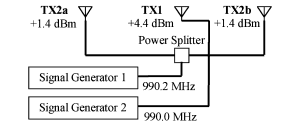
Fig. 4, Transmitter set up simulating Access
Point Repeaters. The total transmitter power from Tx2a and
Tx2b were matched to the Tx1 power.
The transmitter set up is shown in Fig. 4. Simple monopole
antennas were used and were assumed to have an effective gain
of 0 dBi, including matching losses. The uncertainty in the
gain of the antennas resulted in an absolute error in the
path-loss measurements of approximately 3 dB and a differential
error between the single and dual transmitter measurements
of 1dB. The signal strength at each location was measured
using a spectrum analyzer on a trolley, and converted to path
loss by compensating for transmitter power and receiver gain.
A slightly different frequency was used for the single and
dual transmitters to allow simultaneous measurements of the
path loss. The receiver antenna was placed on a rotating platform
that was turned during the received power measurement. Video
averaging on the spectrum analyzer was used to average the
power over a 0.6 m circular path swept by the antenna. This
was done to remove the effects of frequency selective fading,
and obtain an accurate measure of the path loss. Even though
the path loss was measured using a continuous wave transmission,
the results are still valid for a wide-bandwidth modulation
schemes.
Due to limitations of the equipment available, the measurements
were performed at 1 GHz, rather than 5 GHz. This will tend
to reduce the effects of shadowing, as the opacity of most
materials is lower at lower RF frequencies; thus, we would
expect the effectiveness of using APRs to improve at higher
RF frequencies.
V. Discussion of Measured Results
Fig. 5 shows the path loss for the single transmitter. The
path loss increases rapidly with distance from the transmitter.
The path loss in the bottom left corner of Fig. 5 at location
5 x 5 m has a high path loss of over 100 dB, which is equivalent
to 1 km of free-space loss, even though it is less than 20
m from the transmitter. Fig. 6 shows the path loss when two
transmitters were used. In this case the path loss is lower
and much more even.
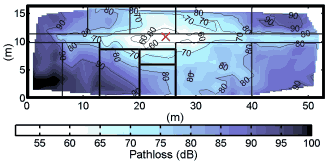
Fig. 5, Measured path loss with one transmitter
over the area of the building at 990 MHz. The dark lines are
the internal walls. The X shows the location of the transmitters.
Fig. 6, Measured path loss for two transmitters
at 990 MHz. This simulates two APRs. Note the total transmitter
power is the same as for the one transmitter measurements.
The X shows the location of the transmitters.
Fig. 7 shows the measured and simulated probability distribution
of the path loss. It shows that the measured path loss is
more than 7 dB lower when using two transmitters as compared
with one. This result is to be expected, as the longest distance
to a transmitter was reduced by 1.62 times. The typical path-loss
exponent of an obstructed path within a building is 4 - 6
[8], as compared to a path loss exponent of 2 for free-space
loss. We would therefore, expect the path loss to be reduced
by 8.4 - 12.6 dB for a reduction in distance of 1.6 times.
Since the transmission power is split over two transmitters
this will reduce the received power by 3 dB, thus resulting
in an overall improvement of 5.4 - 9.6 dB. This compares well
with the measured improvement of 7 dB. Even though there are
two transmitters, the received signal power tends to be dominated
by the closest transmitter due to the rapid fall of power
with distance. This is why the improvement in signal power
can be estimated with reasonable accuracy by considering the
reduction in distance to the transmitter.
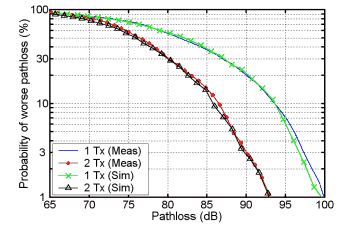
Figure 7, Path loss probability distribution
within the measured and simulated building.
VI. Reduction in Cellular Interference
At a large distance, multiple APRs appear as a point source
and, thus, the received power is the same as when using a
single access point, where the total transmitted power is
the same. This means that the interference to neighboring
systems is approximately the same regardless of the number
of APRs used for fixed total transmission power. The near-field
path loss can be reduced by 7 dB using two APRs, allowing
the total transmitted power to be reduced by up to 7 dB for
the same SNR. This will reduce the overall interference to
nearby WLAN systems by 7 dB. Further improvements could be
made using a higher number of APRs. Using one APR per room
could potentially decrease the near field path loss by 20-30
dB, allowing a large reduction in external interference with
suitable power control. The reduced interference will allow
a better frequency reuse in a cellular system.
VII. Shadow Modeling
A simplified ray trace path loss model was developed to allow
investigation into the use of multiple APRs. This is similar
to the model used in [9]. The two dimensional (2-D) model
calculates the path loss along radial rays, which are converted
to a fixed rectangular data grid using interpolation. The
rectangular grid allows the signal power from multiple transmitter
sources to the added together.
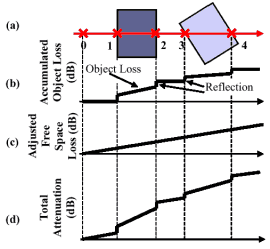
Fig. 8. Attenuation calculations for a ray
passing through two objects.
The path loss along each ray is found by calculating its
intercept to each object in the environment. The loss through
each object is calculated based on the reflection coefficient
of the object surface and the distance through the object.
Each surface the ray intercepts results in energy being reflected
causing a jump in the accumulated attenuation. As shown in
Fig. 8(a), surface 1 - 4 result in jumps in the cumulated
object loss shown in Fig. 8(b). In this model, the amount
of reflected energy is constant regardless of the angle of
the ray. This reflected energy is not calculated as another
ray, and is ignored to keep the model simple.
In addition to reflected losses, each object absorbs energy
as the ray passes through it. This is calculated as a loss
proportional, in dB, to the distance traveled through the
object medium. The loss along the ray is accumulated as the
ray passes through multiple objects. This loss is then added
to the loss calculated by the standard free-space radio path-loss
equation to obtain the overall attenuation with distance.
The radio path-loss equation is
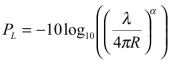
where PL is the path loss (in decibels), lambda
is the wavelength of the radio signal (in meters), r
is the distance from the transmitter (in meters), and alpha
(a) is the path loss exponent. For free space propagation,
a = 2. The simulation calculates the path loss due
to objects and, thus, ideally, the path-loss exponent used
in the simulation should be 2. However due to the simplicity
of the model, a path loss exponent of 2.65 was found to best
match the measured results.
VIII. Model Verification, Tuning and Limitations
The radio model was compared to the measured results to verify
the model accuracy and to adjust the model parameters to give
realistic results. The material properties of the walls were
measured directly and are show in Table I. These values compare
well with values measured in [9]. The walls were found to
be non homogeneous, caused by wiring, reinforcing, studs,
and near by objects, making accurate measurements difficult.
In the simulation, the material properties of the walls were
optimized to obtain a good match between the simulated and
measured building path loss shown in Fig. 5 and Fig. 6. The
resulting simulation parameters were found to closely match
the direct wall loss measurements, as shown in Table I. Fig.
7 shows the resulting match between the measured and simulated
results. This figure shows the percentage area of the building
(Y-axis), which has a path loss worse than a specified path
loss in the X-axis. For example, 20% of the building has a
path loss of greater than 90dB when using a single transmitter,
where as it is only 3% for two transmitters.
There is a good match between the simulated and measured
results, despite the simplicity of the model. If the wall
loss were considerably higher, then the path loss would have
been dominated by diffraction and multiple reflections, resulting
in a poor accuracy in the path-loss prediction.
IX. Simulated Results
Fig. 9 and Fig. 10 show the simulated path loss for a single
and double transmitter respectively, for the same building
and transmitter location as measured data shown in Fig. 5
and Fig. 6. The probability distribution of the path loss
is shown in Fig. 8. The main difference between the simulated
and measured results is that the simulated results are smoother,
which is the result of the simple environment model.
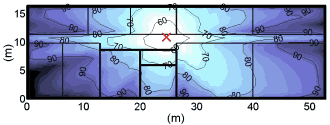
Figure 9, Simulated path loss for one transmitter
at 990 MHz. The X shows the transmitter location.
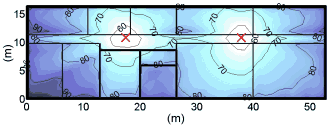
Figure 10, Simulated path loss for two transmitters.
The X shows the location of the transmitters.
In addition to simulating one and two transmitters, the path
loss was simulated with four and eight transmitters. The transmitters
were positioned in the simulation to approximately maximize
their effectiveness. Fig. 11 shows the path loss distribution
for all the simulated results. The path loss for the worst
2% of the area of the building was improved by 7 dB when using
two transmitters, 16 dB for four transmitters and 22 dB for
eight transmitters.
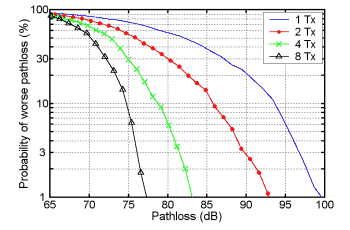
Figure 11, Cumulative probability distribution
for simulated results, showing the result for one, two, four
and eight transmitters.
X. Conclusion
Using multiple APRs is a low-cost method that can be used
to reduce shadowing and near-field path loss within a building.
This allows a reduction in intercellular interference with
suitable power control. It was shown experimentally to decrease
the near-field path loss by 7 dB @ 1 GHz within an indoor
environment, using two repeaters as compared with a single
transmitter. Simulated results show that the improvement can
be as much as 20 dB for eight transmitters. Using multiple
APRs will result in an increase in the received multipath,
however OFDM systems, including Hiperlan/2 and IEEE 802.11a,
should be able to tolerate this without detrimental effects.
This tolerance should allow a maximum spacing of 40-60 m between
any two APRs in a Hiperlan/2 cell.
References
[1] B. Crow, I. Widjaja, J. G. Kim, P. Sakai, "IEEE 802.11
wireless local area networks", IEEE Commun. Mag., pp. 116-126,
Sept. 1997.
[2] HiperLAN/2 - The Broadband Radio Transmission Technology
Operating in the 5 GHz Frequency Band, M. Johnsson, (1999),
[Online]. Available: http://www.hiperlan2.com/site/specific/specmain/specwh.htm
[3] U. Grob, A. L. Welti, E. Zollinger, R. Küng, H. Kaufmann,
"Microcellular Direct-Sequence Spread-Spectrum Radio System
Using N-Path RAKE Receiver", IEEE J. Select. Areas Commun.,
vol. 8, pp. 772-779, June 1990.
[4] HFA3863 data sheet (2000), [Online]. Available: http://www.intersil.com/data/FN/FN4/FN4856/FN4856.pdf
[5] R. Bultitude, S. Mahmoud, W. Sullivan, "A Comparison
of Indoor Radio Propagation Characteristics at 910 MHz and
1.75 GHz", IEEE J. Select. Areas Commun., vol. 7, January
1989.
[6] Digital audio broadcasting - Overview and Summary of
the DAB System, [Online]. Available: http://www.worlddab.org/public_documents/eureka_brochure.pdf
[7] C. Lim, A. Nirmalathas, D. Novak, "Dynamic Range Of A
Multi-Section Laser In A Millimetre-Wave Fibre-Wireless Uplink",
in Proc. Asia-Pacific Microwave Conf., Sydney, N.S.W., Australia,
Dec. 2000, pp. 539-543
[8] The Mobile Communications Handbook. Boca Raton, FL: CRC
Press, 1996, pp. 355-369. [9] S. Y. Seidel and T. S. Rappaport,
"914 MHz path loss prediction models for indoor communications
in multifloored buildings", IEEE Trans. Antennas Propagat.,
vol. 40, pp. 207-217, Feb. 1992.
|